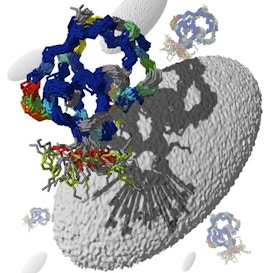
G. Schröder/MPIbpc
Several studies have shown that a micro-duplication within 15q11-13 — a region on chromosome 15 — on the maternal chromosome is associated with autism. The duplicated region contains many genes, but UBE3A, which encodes a ubiquitin ligase, deserves special attention because duplications apparently restricted to the UBE3A gene have been found in people with autism1.
In addition, three heuristic reasons to consider UBE3A come to mind. First, deletions or nonsense mutations of the maternal allele result in Angelman syndrome, a developmental disorder that shares certain features with autism. Inferring effects of a gene duplication based on the effects of a deletion takes courage, but at this stage of our knowledge, the leap is worth taking. I should emphasize from the start that we need studies of ligase overexpression — dose matters.
Second, the maternal UBE3A gene is expressed in several regions of the brain, whereas little or no expression has been detected from the paternal chromosome. This ‘imprinting’ of the UBE3A locus suggests that proper expression of the remaining allele is critical. Third, the UBE3A protein interacts with several proteins implicated in synapse formation and synaptic plasticity, likely to be targets of autism risk factors.
Here, I focus on recent studies of ubiquitin and excitatory synaptic transmission. But first, it is worth considering ubiquitin itself and where it came from.
Ubiquitin, the (un)folding story:
Rudolph Schoenheimer, a German Jewish refugee from National Socialism working at Columbia University in the early 1930s, used stable isotopes of nitrogen to demonstrate that cellular proteins are in a dynamic state. His revolutionary idea — that there is a delicate balance between protein synthesis and protein degradation — was described in his Dunham Lectures, published in 1941, one year after his death by suicide.
Thirty years later, Avram Hershko showed that protein degradation is an active process that requires energy in the form of ATP. At about the same time, Gideon Goldstein, searching for factors that promote the differentiation of lymphocytes — key players in the immune system — isolated an 8 kilodalton polypeptide2. He named it ubiquitous immunopoietic peptide (UBIP) because he found it to be ubiquitously distributed in animal tissues and in plants, and because it affects a broader population of cells than he expected.
Over the next decades, Hershko, Aaron Ciechanover, Alexander Varshavsky and their colleagues demonstrated the importance of the 8 kD peptide, now known as ubiquitin. Their remarkable studies identified enzymes that catalyze the ATP-dependent activation of ubiquitin (group E1), that promote the formation of poly-ubiquitin chains (group E2), and a third class (group E3) that catalyze the ligation of ubiquitin to protein targets. The specificity of this ligation step is crucial, as it tags proteins for degradation, recycling or redistribution within the cell. Informative reviews of the ubiquitin system by the three authors appeared ten years ago3.
It is not easy for a newcomer to trace the origin of the name ‘UBE3A.’ Here is my understanding.
Peter Howley at Harvard University and colleagues, in the course of their studies of cervical cancer, purified a ubiquitin ligase on the basis of its ability to bind to the E6 protein of papilloma virus, the causative agent4. They named it the E6 associated protein, or E6-AP. This ligase has actions beyond cervical cancer and recognizes many targets in addition to the E6-AP. In many circles, it has become known as UBE3A.
We now know that the human genome contains more than 600 ubiquitin ligase genes. UBE3A is a member of a small subset named HECT domain ligases, short for homologous to the E6-AP carboxy terminus. One of the most important and productive areas of ubiquitin research involves the identification of relevant targets for ligase enzymes.
HECT ligases:
Four important papers published in the past six months suggest that HECT ligases influence the formation, stability and function of excitatory synapses.
In the first of these, Ben Philpot and his colleagues at the University of North Carolina and Duke University reported that the UBE3A locus is indeed imprinted in the mouse visual cortex5. They found that the frequency of miniature excitatory postsynaptic currents (mepscs) recorded in layer 2/3 pyramidal neurons increases during postnatal development of control mice, but does not increase as much in UBE3Am-/p mice over the same period of time.
Mepscs reflect the spontaneous release of neurotransmitter packets from nerve terminals. Assuming a constant frequency at each synaptic contact, the overall mepsc frequency can be taken as a measure of the number of synapses – as a first approximation. There was no difference in the amplitude of mepscs during the same interval.
The combination of a smaller increase in mepsc frequency with no change in mepsc amplitude suggests that UBE3A influences the formation or maintenance, rather than the efficacy, of individual synapses. In fact, the density of spines, loci of excitatory synapses, along the basal dendrites of pyramidal neurons in the visual cortex was reduced.
Philpot and his colleagues also found little or no increase in mepsc frequency when wild type animals are reared in the dark. The low mepsc frequency in UBE3Am-/p mice is not further reduced by rearing the animals in the dark5. What’s more, the expected shift in ocular dominance does not occur when one eye of aUBE3Am-/p mouse is deprived of light. Long-term potentiation and long-term depression are both lower in UBE3Am-/p+ mice.
Taken together, these findings point to a deficit in activity-dependent synaptic plasticity. The researchers did not discuss UBE3A targets that might be relevant mediators.
It is noteworthy that late-onset visual deprivation, beginning 21 days after birth, restores synaptic plasticity in the absence of UB3EA. Recall that behavioral deficits in MeCP2 knockout mice, the reigning model of Rett syndrome, are reversed when the gene is activated in mature mice6. Mechanisms that might explain the reversals are not clear in either case, but both studies present hopeful signs developmental deficits can be overcome later in life.
A study of hippocampal pyramidal neurons, published by Michael Greenberg and colleagues, also points to an activity-dependent role for UBE3A in regulating excitatory synapses. In this study, potassium-induced membrane depolarization or glutamate receptor activation are taken as surrogates for neural activity.
They also found a decrease in mepsc frequency, without a change in mepsc amplitude, in UBE3Am-/p mice. They concluded that the decrease in mepsc frequency is a result of the appearance of silent synapses, rather than because of a loss of synaptic contacts. The researchers did not detect any change in the dendritic arbor of the pyramidal neurons, and the number of GluR1 containing ‘puncta’ is not decreased. The net effect is the same as that reported by Philpot and his colleagues, but the mechanism may be different.
Greenberg’s group raised the interesting possibility that ARC is a target of UBE3A. ARC stands for activity regulated cytoskeleton-associated protein, and several studies indicate that ARC regulates AMPA-type glutamate receptor trafficking.
The researchers arrived at ARC via a database search for proteins that contain a 75 amino acid stretch, which they propose as a consensus ubiquitin-binding domain (UBD), an interesting possibility that needs further documentation.
However, it is clear that UBE3A leads to the ubiquitination of ARC. What’s more, levels of ARC are higher in UBE3Am-/p mice. Their thinking goes like this: activation of AMPA receptors up regulates ARC expression, followed by down regulation of AMPA receptors. UBE3A-catalyzed ubiquitination of ARC leads to degradation of ARC, interrupting the protective negative feedback loop, and leading to excessive excitation.
NEDD news:
Two other studies deal with a different HECT ligase, called NEDD 4.1.
Nils Brose, Hiroshi Kawabi and colleagues found that hippocampal neurons dissociated from NEDD 4.1 knockout embryos, and maintained in sparse cell cultures, are smaller than neurons from wild-type mice7. Their neuritic arbors are also less complex than those of controls.
They found that the mepsc frequency is reduced with no change in mepsc amplitude, and the number of synaptic boutons (autapses) is also reduced. They concluded that ubiquitination of certain proteins is involved in regulating the number of excitatory synapses.
Sound familiar?
The Brose group identified potential targets of NEDD 4.1 by affinity chromatography and immunoprecipitation. Their attention was drawn to a tyrosine kinase named TNIK because earlier studies had linked this kinase to actin dynamics, a key determinant of neurite branching. They provided evidence that TNIK links NEDD 4.1 to RAP2, a GTPase involved in receptor recycling. RAP2 is the member of the complex that becomes ubiquitinated.
In the same issue of Neuron, Christine Holt, Jovana Drinjakovic and colleagues reported that blocking NEDD 4.1 activity in the retina inhibits the branching of retinal axons in the optic tectum8. NEDD 4.1’s role appears to be restricted to growth cones at the end of their journey, as the course of ganglion cell axons in the optic nerve was undisturbed.
Based on a review of the literature, the Holt group focused on PTEN as a relevant target of NEDD 4.1. PTEN is present in ganglion cell axons, it is a target of NEDD 4.1, and it regulates PI3K, an enzyme that has several effects on axon turning and neuronal size, via effects on the cytoskeleton.
In summary, there can be little question that UBE3A and other HECT ligases have profound effects on the number of functional excitatory synapses in the mouse brain. Among the most pressing issues are the delineation of immediate and relevant ligase targets, where they act in the brain, and when their effects are first manifest.
Another key issue concerns the relation between simplified models of neural activity studied to date, and the ongoing patterned activity in the developing brain. Further, we must understand how changes in synaptic structure and function influence patterns of activity in local circuits that are relevant to thinking and behavior associated with autism.
These are key issues that must be raised for all autism risk factors. Indeed, they are among the most profound issues in all of neuroscience.